All life on earth, blue whales, rabbits, ants, bacteria, even TSA agents, is electrically powered. The powerhouse of the cell is just a warehouse where countless high-power electrons discharge energy through thousands of nanometre-scale generators. We keep these generators spinning1 by molecularly incinerating the food that we eat to release more high-power electrons.
Harnessing electrons is so universal to life that some scientists even posit electron flow as a prerequisite to life’s origin. Living systems eat energetic electrons trapped in molecules and then literally burn them to extract power. While oxygen makes for great fires, life is so addicted to this process that it will make do with whatever “electron acceptor” it can find. If you’ve ever built a Winogradsky column, then you’ve seen this principle in action. But life isn’t picky about where the energized electrons come from either. Sunlight, sugar, hydrogen gas, actual electrodes, maybe even radioactive decay – it all works.
It seems as though life will “colonize” any suitable source of nutritious electrons, and we call this seeming “microbial infallibility”. So I ask: given that mini fridges exist, where is all the heat-powered life?
On Earth, most electricity is made by turning an axle. Wind, water, and steam from coal fires and nuclear fission are all cajoled into rotating a generator and inducing a current. In space, we tend to want to limit the number of moving parts and so rotating generators aren’t really the mode. Instead, we either sun-roast electrons off semiconductors (solar panels), or use batteries powered by red-hot plutonium. These “radioisotope thermoelectric generators” exploit a heat differential between the radioactive metal and the inhospitable cold of space to induce a modest, but reliable electrical current without any moving parts. I wonder if there are organisms that do something similar (but maybe without the plutonium).
This “thermoelectric effect” is not just relegated to multi-million dollar space missions, it’s also found in hotel rooms around the world. Beyond its mystical ability to triple the price of any snack it contains, the hotel mini fridge also manipulates electrons in a way that is hitherto unknown to biology. By passing electrical current through a plate of two conductors, the “Peltier effect” leads to the build up of heat on one side, and the cooling of the other – a quiet way to cool a box of treats. And the crazy thing is that if you instead provide the heat and the cold to the plates, you’ll receive a current out of its wires. A change in heat can directly give you voltage.
Much to the chagrin of my undergrad self, Anthonie Muller first proposed that life could be driven by “thermosynthesis” over a decade before my birth2. Questioning if the boring sunlight and sugars we’ve known about for centuries were the only vital forces on Earth. Muller proposed multiple models for how a biological “heat engine” could be built over the ~8 nanometre outer membrane of a cell. While fascinating, these ideas all rely on a gradient forming over a tiny distance – just 30 water molecules end-to-end. I’m no entropist, but that doesn’t feel workable to me. But if we take the long view – I think there’s something here.
A thin Peltier module can be ~0.5 millimetres thick, so we’re looking for naturally occurring half-centimetre biological wires. Enter the star of our speculative science fiction: Desulfobulbaceae – better known as Cable Bacteria. As their moniker suggests, Cable Bacteria grow in chain-shaped colonies that easily exceed our threshold, reaching lengths of 15 mm. But unlike other “filamentous” microbes, Cable Bacteria chains are electrically conductive. In fact, they rely on this conductivity to short-circuit aquatic sediments to steal oxygen from layers that they wouldn’t normally have access to. Cable Bacteria keep their lights on by shunting their electrons from, microbially speaking, low prestige sulfidic sediments into snooty, oxygen-rich layers by physically bridging these layers with their Cable. So my question is, what happens if one side of one of these Cables gets warm?
Let’s say we have a little microscopic forest of Cable colonies at the bottom of a geologically active alpine lake, happily redistributing the oxidative wealth. The lake water is cold, keeping the highest, oxygen-rich layers chilled. But the sediment has built up on the geologically warmed lakebed, so the sulfidic base is routinely warmer. Could our Cables experience an electrical bump from this temperature differential? I have no clue. The best I understand it, a Peltier module needs two different conductors, do the Cable’s different conductive cytochrome proteins satisfy this requirement? Maybe? And in the best case, let’s say there’s a 50 °C temperature differential over 1 cm, how many electron volts could we hope to draw? Could that power, or at least supplement bacterial life? I wouldn’t know. If you have an idea on how to calculate these ballparks, I’d love to hear your thoughts in the comments!
It turns out that dictating the flow of electrons is a lucrative endeavor: often, electrical engineers restrict a current’s flow to a particular direction by using a one-way door called a “diode”; the brain that you’re using to read this blog can only function because of unidirectional biological wires called “neurons”; and disrupting how charge segregates in the mitochondrion is one weight loss trick that doctors definitely don’t want you to know about. The same is probably true for our Cables. They shunt electrons up through the sediment to live, but what if another electrical pressure pushed those electrons down the other way? What if a Cable, that can experience a thermoeletric effect, is arranged the wrong way in the thermal gradient? I’d imagine this would be no good. So given the existence of diodes, and one-way neuronal conduction, and the mechanisms in place to prevent mitochondrial “decoupling”, I’d posit that if Cables can experience a thermoelectric effect, I would also expect them have diode-like systems in place to deal with disruptive thermoelectric current. Further, Cables can move through bacterial gliding, so I’d also expect them to try and optimally align themselves to thermal gradients.
Our complete transition to renewable energy sources like solar is in part being stopped by a duck. The “duck curve” results from the uneven rate at which solar energy is produced versus its consumption. During the day, when electrical lighting is least needed, we generate the most solar electrical energy, but we’re not too good at storing that energy for later use in the evening. What I’m driving at is that to efficiently use power from environmental sources, one needs to be able to rapidly adapt to changes in production efficiency. Sometimes it’s really bright and if we don’t act, we waste that overproduction, maybe even damage our power grid. Other times it’s too dim and we better hope we made use of our previous brighter boon times. The same issues exist in microbial energetics. An equation has to be continually balanced between production and consumption. Fail to do so and at best you’re being inefficient, but at worst you’re accumulating toxic energetic byproducts or unnecessarily starving during future badtimes. So with thermal gradients being hard to predict, I’d expect thermoelectric Cables to have machinery to react to electron gluts and dearths caused by fluctuating thermal gradients.
All of this speculative navel gazing is to say, if Cable Bacteria are sensitive to thermoelectric effects, I’d expect to observe these three biological features:
- Cables will grow better when productively aligned with a thermal gradient
- Cables will grow worse when perfectly mis-aligned with a thermal gradient
- Given enough time to respond, Cables should be able to optimize for growth rate in sub-optimal thermal gradients
This sets me up to propose a test for thermoelectric behaviour in Cables: In a square culture flask, establish a classical Cable community. Then, mount heating/cooling elements below and above the community (also mount elements on the side-to-side axis as a control gradient). Measure Cable growth rate by microscopy ,or rate of carbon fixation, or hydrogen sulfide oxidation – whathaveyou. Set up Cable co-axial thermal gradients with the vertical elements, keeping the average temperature fixed, and staying within a range that limits frying/freezing the bugs. Measure growth rate and see if it differs from a perfectly off-axis control gradient. Such an experiment might look something like this:
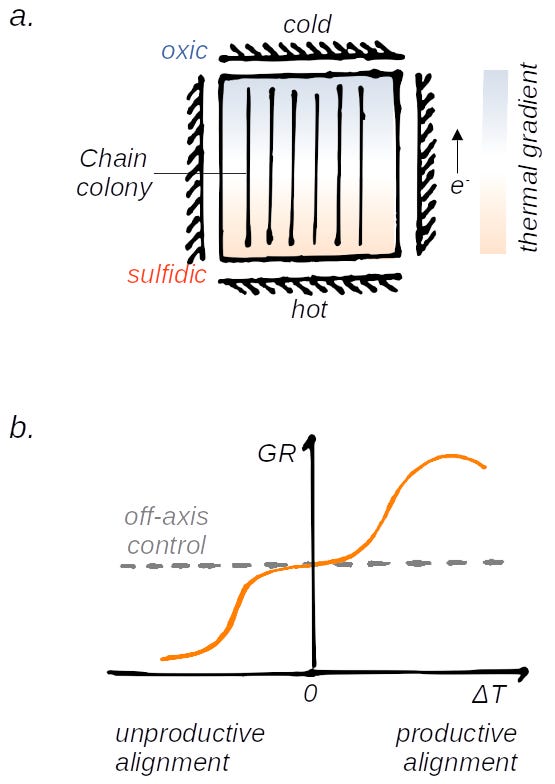
There are of course, loads of caveats. Chains will actively respond to their environment to make their lives better, so the observed response to thermal gradients will vary with how much time a Cable has had time to respond. A Cable might throttle its growth to prevent burning out, or it might start synthesizing ‘diode genes’ to deal with toxic electric current. And the response might not follow the logistic curve I’ve instinctively gone for. All I want to say is that I think there’d be different responses depending on the orientation, duration, and magnitude of an applied thermal gradient.
So what’s the point of all this? Firstly, I am fascinated with life’s inventiveness. Thermal gradients might be the oldest form of energetic passive income out there, so I think it’s worth asking if life has found a way to use them. I’d even go so far to suggest that there might even be a fossil record of such a lifestyle. We might find that ancient bacterial colony fossils might have a conspicuous alignment with/against ancient thermal gradients. Such a finding would re-write our history of life on Earth. But there are also potential biotechnological applications. We lose so much power to heat. What if our roofs were coated in domesticated Cable mats that could fix carbon from our atmosphere using our lost radiated heat? What if our dirty engines could sport a Cable velvet that produces pharmaceutical products while we burn gas? Or what if the electrical genes found in these Cables could be used in future efficient, and self-healing material technologies? I think all these prospects make this wacky idea worth looking into. At the very least, we might get a more efficient mini fridge.
Originally published on substack
This is an award-winning entry for the Ideas Writing Challenge